As the most common chronic lung disease in infants, bronchopulmonary dysplasia (BPD) is associated with long-term sequelae that extend into adulthood. Despite significant improvements in perinatal care, the incidence of BPD has remained unchanged or even increased among the most immature infants. The variation in incidence of BPD across perinatal centers reflects differences in both patient population and treatment modalities.
As the most common chronic lung disease in infants, bronchopulmonary dysplasia (BPD) is associated with long-term sequelae that extend into adulthood [1,2]. Despite significant improvements in perinatal care, such as prenatal treatment with steroids to achieve a maturation “spurt” of the lungs, surfactant therapy, and the development of adapted ventilation strategies, the incidence of BPD in the most immature infants has remained unchanged or even increased [3]. This is likely due to a significant reduction in mortality rates along with an increase in the total number of treated infants born significantly premature. The variation in incidence of BPD across perinatal centers reflects both differences in patient population and treatment modalities [4–7]. Publications report an incidence of BPD of up to 68% in very low birth weight infants (401-1500 g) with a gestational age less than 29 weeks’ gestation, or up to 77% in infants born at less than 32 weeks’ gestation or with a birth weight less than 1 kg [5,8,9]. These figures come mainly from countries with a high gross domestic product. With approximately 15 million children born prematurely each year worldwide, the above figures demonstrate the significant clinical and socioeconomic challenge [10].
Chronic lung disease of the newborn is classified into three severity levels as defined by Jobe and Bancalari: mild (oxygen supplementation for at least 28 days postnatally), moderate (oxygen supplementation <30% at 36 weeks postmenstrual age), and severe (oxygen supplementation 30% and/or ventilatory support at 36 weeks postmenstrual age) [1].
Large clinical trials have identified numerous risk factors for the development of BPD, including congenital and nosocomial infections, mechanical ventilation, and oxygen toxicity [11–16]. The impact of these prenatal and postnatal challenges is further determined by the presence of caloric deficiency or deficiency of vitamins and trace elements, as well as inadequate secretion of adrenal and thyroid hormones, which further increase the risk of developing pulmonary morbidity [17–19]. The vulnerability of the developing lung to the development of chronic damage is also increased 3 to 4-fold by the presence of intrauterine growth retardation [20–24], with alveolar and vascular development being critically affected by the underlying altered signal transduction [25]. Exposure to prenatal cigarette smoke – widely underestimated clinically due to lack of anamnestic information and clinical markers – has also been shown to contribute significantly to disease development, possibly beyond an impact on somatic growth [26,27].
The role of established therapies also needs to be kept under review with regard to a possible contribution to the development of chronic complications. Effects of steroid treatment and maternal antibiosis are discussed below in light of this.
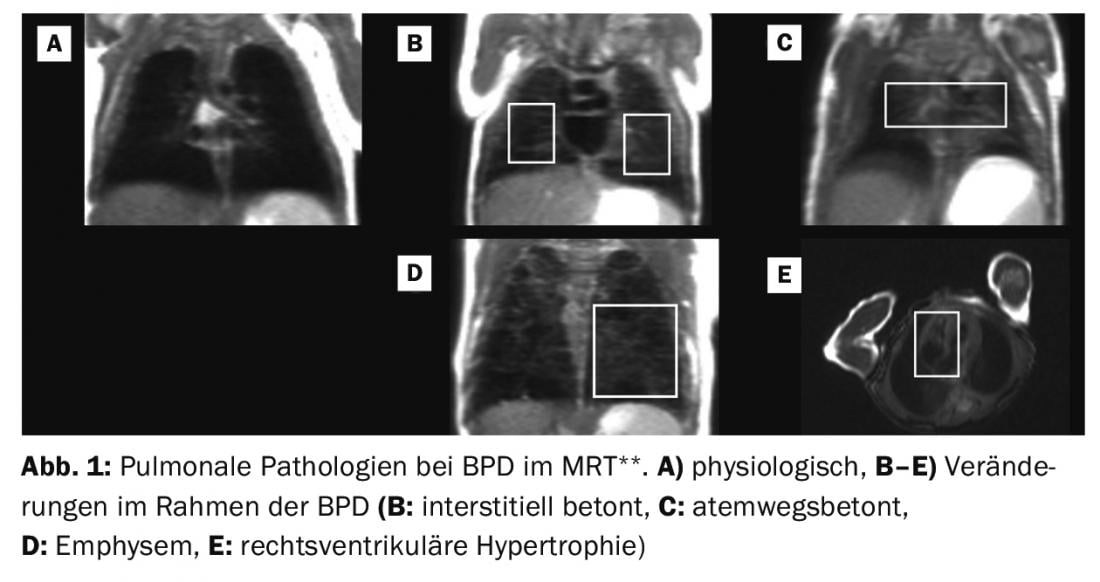
** Adapted from: MRI based scoring of the diseased lung in the preterm infant with BPD. Kai Förster, Hannah Busen, Sophia Stöcklein, Olaf Dietrich, Harald Ehrhardt, Mark O. Wielpütz, Andreas W. Flemmer, Benjamin Schubert, Marcus A. Mall, Birgit Ertl-Wagner, Anne Hilgendorff. This manuscript is under submission at Thorax.
Finally, the discussion on the importance of different risk factors has to be conducted in the light of our current, constantly evolving knowledge on the importance of genetic polymorphisms, and studies report that up to 53% of the variance in BPD may be due to this [28]. Genetic abnormalities identified include mutations in genes associated with surfactant synthesis, innate immune response [29,30] and superoxide dismutase [31]. The higher risk of developing BPD and pulmonary arterial hypertension (PAH) in preterm male infants [32] has been linked to differences in hormonal regulation [33]. In contrast, further down the line, women with a history of BPD are more affected in the long term [34].
Difficulties in identifying clinically relevant genetic risk factors arise both in differentiating between variables influencing the risk of preterm birth per se [35] and in considering acute [36] and chronic complications in relation to each other. However, the fact that large genetic association studies [37] have so far not been able to follow up on the successes in other disease areas such as cystic fibrosis [38] or PAH [39,40] in terms of landmark results with clinical relevance may, in addition to the reasons mentioned above, also be an indication of the heterogeneity of BPD diagnosis. In the future, identification of underlying disease (sub)entities may allow clearer assignment of specific risk factors and genetic polymorphisms. Similarly, knowledge of key sites in signal transduction that control the interplay of different pulmonary cell populations will enable the classification of candidate genes. The following sections address various aspects of this.
From cause to consequence: inflammation and oxidative stress response
The described exogenous influences acting pre- and postnatally on a structurally and functionally immature lung result in a persistent inflammatory response, remodeling of the extracellular matrix (ECM), and diffuse fibrotic changes including smooth muscle hypertrophy in the small pulmonary arteries and airways [41]. From the characteristic histopathological changes with the picture of disturbed alveolarization and vasculogenesis [1] results alveolar hypoventilation, which is expressed in the clinical picture of hypercapnia and hypoxemia and the ventilation-perfusion mismatch [42].
The acute and chronic inflammatory processes that characterize BPD are caused by both pre- and postnatal mechanisms. Infections and the corresponding ability to generate a competent immune response play an important role in the development of BPD [13,43–45]. Prenatal inflammatory processes, summarized for example by the term “fetal inflammatory response syndrome (FIRS)” or occurring in the context of manifest infectious events such as chorioamnionitis prenatally or postnatally in the context of congenital and nosocomial infections, lead to an influx of neutrophilic granulocytes into the immature lung. As a result, there is the presence of increased numbers of monocytes and macrophages as part of the so-called “second wave” of the immune response [24,46,47]. The role of innate immunity is of particular importance here, as adaptive immunity can also be variably expressed depending on gestational age [48]. Animal studies suggest that extracellular matrix remodeling and early alveolar epithelial dysfunction are not only a consequence of the inflammatory response, but also further promote it [49,50], causing lasting changes in pulmonary immune function. Prenatally, the widespread use of maternal antibiotic treatments leads to a lasting change in the bacterial flora in the child [51] and in the immune function of the offspring in a mouse model [52].
Postnatally, the induction of barotrauma and volutrauma injuries during mechanical ventilation and the consequences of moderate or severe hyperoxia are important risk factors for the initiation and maintenance of the inflammatory processes highlighted above at the local and even systemic level [53–56]. The release of cytokines such as transforming growth factor (TGF)-β, tumor necrosis factor (TNF)-alpha, and interleukins, e.g., IL-1beta, contributes significantly to the imbalance of signal transduction of various (other) growth factors and leads to the activation of transcription factors that promote apoptosis in different cells [57–59]. Discussion of the role of recruited and resident inflammatory cells [60–64] will provide important insights into mechanistic relationships and for identifying therapeutic options. In this context, however, the presumed influence of inflammatory cells on lung development will also generate new avenues of research [65].
Acute and chronic inflammatory processes must be considered in the context of the cellular capacity to respond to persistent or recurrent postnatal challenges. Here, the relative lack of antioxidants and inhibitors of proteolytic enzymes makes the immature lung particularly vulnerable to the effects of toxic oxygen metabolites and released proteases from the extracellular matrix and resident or recruited neutrophil granulocytes and macrophages [66-69]. Several studies have demonstrated evidence for increased oxidative stress in the preterm infant. Thus, urinary malondialdehyde concentrations are elevated in the first week of life, resulting from peroxidation of lipid membranes after oxidatively mediated damage, and have been shown to correlate with risk for oxygen radical diseases, including BPD [70]. Decreased concentrations of pulmonary antioxidants have been measured in the lavage of preterm infants [71], and other studies indicate that juvenile BPD pa-tients are characterized by signs of increased oxidative stress in the airways, a sign of long-term changes in the respiratory system after preterm birth [72]. Established therapies also need to be critically reviewed for their role in pulmonary development in light of new evidence, as, for example, prenatal administration of betamethasone, despite its more widespread prenatal use to promote lung maturation and prevent respiratory distress while decreasing rates of BPD [73,74] Have been shown to increase indicators of lipid membrane peroxidation [70].
These longer-term changes in the oxidative stress response and other processes are reflected in altered responses to viral infections later in life [75]. The response of the developing lung to early damage, including long-term consequences, is specific and different from the response of the adult organism. Whereas chronic exposure to oxygen (60% for 14 days) in neonatal rat lungs increases contraction of pulmonary vessels and airway smooth muscle and reduces nitric oxide relaxation, the opposite phenomenon occurs in adult animals [76]. Long-term effects after hyperoxia exposure in the first week of life (100% for 4 days) include primarily cardiovascular complications, in which right ventricular strain developed as a consequence of pulmonary vascular disease in PAH and consecutively increased mortality in animal models [77]. Altered signal transduction by bone morphogenic protein (BMP) offers a pathomechanistic explanation. Other mechanisms explaining the increased susceptibility to acute and long-term damage in the newborn lung are demonstrated by another study of hyperoxia-induced effects. In the neonatal mouse, in contrast to the adult animal, bone marrow-derived circulating and lung endothelial progenitor cells are markedly reduced here [78], which may result in early exhaustion of repair and regenerative capacities. The impact on other key processes such as cell cycle regulation with upregulation of P21 after hyperoxia and reduced histone deacetylase activity [79] as well as the effects on DNA methylation [80] also point to the early emergence of long-term relevant accumulative effects in the context of reduced compensatory mechanisms in the immature lung.
Histopathological characteristics – clock and “memory” function
The interaction of the epithelial, mesenchymal, and endothelial cell compartments in their interplay to develop the gas exchange area is largely orchestrated by the regulation of various growth factors. These include Notch and Wingless Int-1 (Wnt), fibroblast and platelet derived growth factor (FGF and PDGF), and BMP and vascular endothelial growth factor (VEGF) [81–87]. Early interference with these transcription factors disrupts normal lung morphogenesis [88] resulting in a lack of differentiation of alveolar structures [89]. Similarly, regulation of key transcription factors such as nuclear factor kappa B (NF-kB) plays a role and contributes to the maldevelopment of the gas exchange surface [88,90,91]. The characteristic association of impaired alveolarization with the presence of dysmorphic capillaries is driven by altered angiogenic growth factor expression, which includes reduced pulmonary expression of vascular endothelial growth factor (VEGF) and VEGF receptors [92–94] as well as decreased endothelial nitric oxide synthase (eNOS) and soluble guanylate cyclase (sGC) in pulmonary blood vessels and airways include. [95,96]. The changes are similar to those in the aging organism and contribute – also due to the reduced plasticity of capillaries and small vessels – to the risk of PAH and impaired development of the lymphatic system in the lungs in the further course [97–100].
The impaired signal transduction together with direct effects of, for example, the stretch stimulus from mechanical ventilation and oxygen toxicity contribute to severe changes in the lung scaffold [101,102]. Increased remodeling of the extracellular matrix is indicated, for example, by increased urinary excretion of desmosine, which is preceded by increased elastase activity [103–105] and is similar to findings in patients with acute respiratory distress syndrome (ARDS) [106]. However, physiological lung development also depends on the presence of pulmonary elastases and metalloproteinases, as complete matrix metalloproteinase deficiency promotes BPD-type lung remodeling [107].
As a result of remodeling, BPD is characterized by pathological arrangement of elastin and qualitative and quantitative changes in the collagen skeleton. [108–110] characterized, which influence the structuring function of the matrix as a scaffold for the formation of new alveoli and capillaries and define the fate of the cells that populate the developing organ [111,112] (Fig. 2). The irreversible reorganization of the extracellular matrix will thus lead to long-term changes that will manifest themselves at various levels.
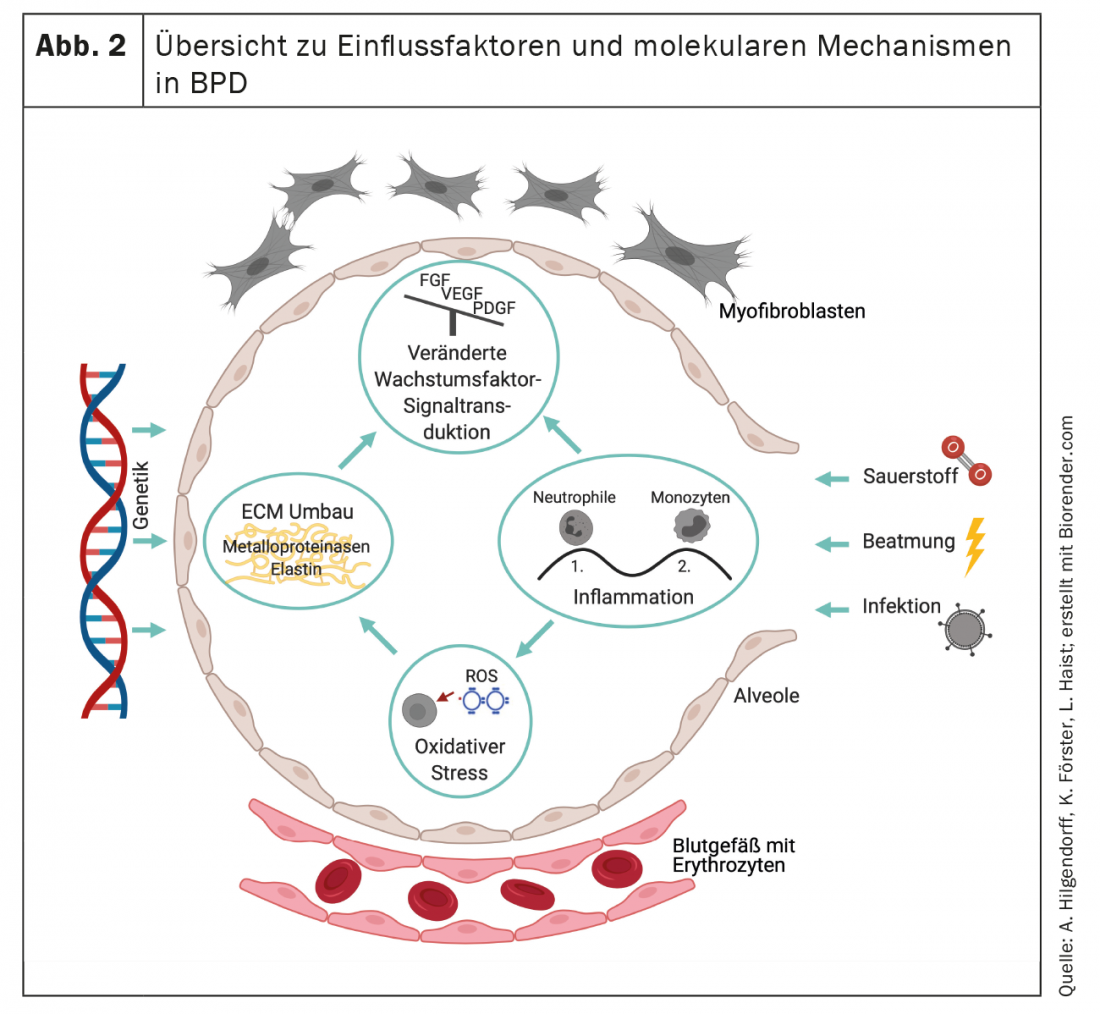
The histopathological alterations and the clinically heterogeneous development and appearance of BPD suggest the existence of disease (sub)entities or at least an individually different influence of various structural alterations on the clinical appearance and long-term course. The presence of primary emphysematous or interstitial remodeling processes, vascular changes and/or pathologies of the respiratory tract are presumably directional “variables” of BPD, which can also be depicted image-morphologically in premature infants by means of clinically feasible imaging strategies in initial studies [113–117]. With the replication of successful studies from other disease areas, the use of additional imaging strategies [118–121] could further improve patient stratification in the future.
Lung function in BPD – Early changes and long-term consequences.
Despite sustained efforts in perinatal care to prevent long-term complications [122,123], manifestation of respiratory symptoms in adulthood is common and often misinterpreted as asthma or COPD, especially when early life events are not known or inquired about [124,125].
Clinically, pulmonary dysfunction is characterized by decreased pulmonary compliance, tachypnea, and increased minute ventilation reflected by increased work of breathing with and without oxygen dependence. This clinical picture may be accompanied by an increase in microvascular pressure in the lungs, which contributes to the development of interstitial pulmonary edema. The increased pulmonary vascular resistance, typically associated with decreased responsiveness to inhaled nitric oxide and other vasodilators, may progress to reversible or persistent PAH and right heart failure [99,100]. Knowledge of early changes in lung function is critical because more severe lung disease after birth is more likely to develop into moderate/severe BPD on the calculated date of delivery [126] and lung function is a good predictor of subsequent morbidity and mortality [127]. BPD is characterized in lung function by increased airway resistance and hyperreactive airways [128]which manifests clinically – in association with or independent of pulmonary infections – by episodic bronchoconstriction and cyanosis (Fig. 3). The proportion of vascular-mediated pathology involved in this beyond the Euler-Liljestrand effect often remains unclear because sensitive indicators are lacking. Affected infants may remain oxygen dependent for months or years, with only a minority actually dependent on oxygen beyond the age of two years [129,130]. Oxygen dependence distinguishes the particular severity of lung disease, and these infants are twice as likely to be readmitted to the hospital compared with nonoxygen-dependent infants. However, even after cessation of oxygen therapy, patients with moderate or severe in up to 70% require repeat hospitalizations, especially in the first two years of life [126,131,132]. Overall, however, lower respiratory tract infections caused by respiratory syncytial virus (RS) remain the leading cause of hospital readmission in preterm infants regardless of BPD status [133].
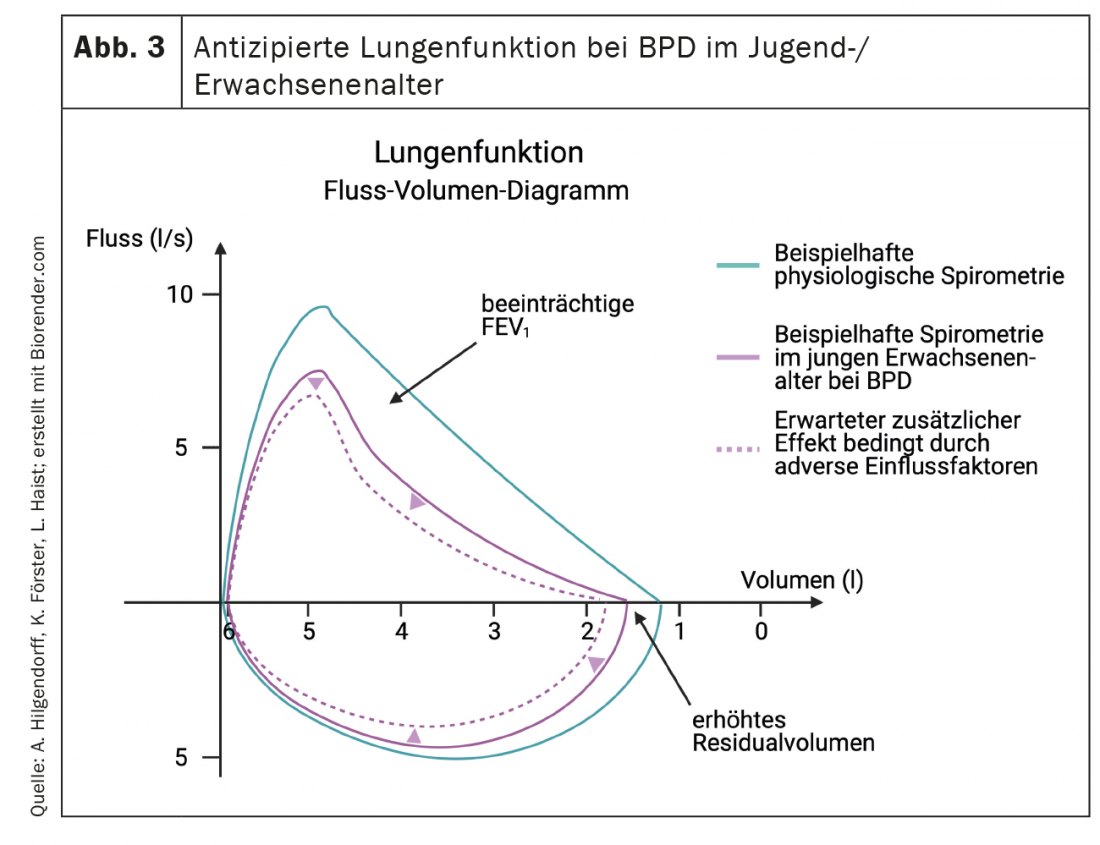
Later in the course of the disease, BPD is a significant risk factor for persistent “wheezing” and for the need for inhalation therapy (odds ratios 2.7 and 2.4, respectively), which affects approximately 20-30% of infants with BPD at six and 12 months of age [134,135]. Respiratory symptoms, especially in children with a history of wheezing, often persist through preschool and school age [129,136] and up to 80% of preterm infants present with frequently symptomatic airway obstruction in early childhood and adolescence [137–139]. Important data on long-term outcome after preterm birth were obtained in the EPICure study [140]. Preterm infants with extreme immaturity suffer significantly lower peak oxygen consumption (“PEOC”) at school age as a direct assessment of cardiorespiratory fitness, lower forced expiratory volume at one second (FEV1), and impaired gas transfer. Here, significantly lower peak loads and higher respiratory rates combined with lower tidal volumes during peak loading and increased residual capacity may reflect the effect of hyperinflation on airway obstruction and/or changes in pulmonary chemoreceptor function and indicate the presence of persistent limitations in airway function and reduction in alveolar area. The non-physiological lung growth based changes with impaired FEV1 and FEV1/forced vital capacity in the context of BPD have been confirmed by several studies in children and young adults [141–144].
The course of the disease into adulthood varies. In some patients, particularly those with severe BPD, symptoms persist into adulthood [145]. Other courses indicate a transient (subjective) improvement with a subsequent recurrence of disease symptoms that are the result of (further) limitation of lung function below a clinical (or individual) threshold. This reduction may appear due to aging processes or an emerging mismatch in lung-to-body mass ratio and/or energy expenditure. While one group reported a divergent course of lung growth during adolescence according to spirometric measurements [141], the EPICure study showed no catch-up of suboptimal lung growth from 11-19 years in adolescents after extreme prematurity regardless of BPD status and even demonstrated significant impairment of all lung function parameters in 19-year-old extremely premature patients [144]. Meanwhile, Vollsaeter and colleagues reported parallel courses of lung function in early adulthood [146].
To better understand features and effects of premature pulmonary aging after prematurity. [147,148] and to clarify the characterization of the effects of secondary injury, e.g., from smoking, from exacerbations in the setting of viral infections, and from broader environmental influences on the course of lung function [149,150] will also be critical for early counseling of families with an eye toward long-term outcome and agreement on control strategies.
Another field is defined by the occurrence of comorbidities for which BPD is a risk factor in its own right [151]. This concerns neurological and cardiovascular diseases and includes consideration of therapy-associated complications. Here dexamethasone treatment, in particular, takes on a special role with adverse effects on cardiac function, life expectancy and neurological development [152,153].
Summary
The response of the immature lung to pre- and postnatal damage mechanisms that manifests as a sustained inflammatory response, an oxidative stress response, and altered growth factor signal transduction, leading to severe alterations in alveolar and vascular development including remodeling of the extracellular matrix, is associated with significant long-term consequences. Abnormalities of lung function (and immunity) in infancy and early childhood predispose to reduced lung function in adult survivors of preterm birth and alteration of the physiologic aging process through a pulmonary “memory effect” in response to early injury. Iterative knowledge exchange between pediatricians, family physicians, and adult pulmonologists is needed to generate a better understanding of these long-term outcomes, including the characteristics and effects of premature aging, and translate them into adapted treatment strategies and lifestyle recommendations for this patient population. In the context of the age at which BPD was diagnosed, interpretation of the pulmonary function findings should also take into account the original underlying BPD definition and the standards of perinatal care applied.
Take-Home Messages
- BPD is a chronic respiratory disease of infancy characterized by alveolar rarefaction, small airway affection, and pulmonary vascular changes. During follow-up observations of pulmonary function, one second capacity may be decreased in children and adults with a history of BPD, among others.
- The characteristic association of impaired alveolarization with the presence of dysmorphic capillaries is driven by altered expression of angiogenic growth factors. Some changes resemble those in the aging organism and contribute to the risk of PAH and impaired development of the lymphatic system in the lungs as the disease progresses.
- As a result of remodeling, BPD is characterized by a pathological arrangement of elastin and qualitative and quantitative changes in the collagen scaffold, which affect the structure-giving function of the matrix as a scaffold for the formation of new alveoli and capillaries and define the fate of the cells that populate the developing organ.
- Early damage to the lung results in a sustained inflammatory response, an oxidative stress response, and altered signal transduction of important growth factors.
- Iterative knowledge exchange between pediatricians, primary care physicians, and adult pulmonologists is necessary to achieve a better understanding of long-term outcomes, including the characteristics and effects of premature aging.
- The impact of early chronic lung disease on the development of comorbidities must be considered when designing monitoring and treatment strategies and making lifestyle recommendations for this patient population.
Literature:
- Jobe AH, Bancalari E: Bronchopulmonary dysplasia. Am J Respir Crit Care Med 2001; 163(7): 1723-1729.
- Duijts L, van Meel ER, Moschino L, et al: European Respiratory Society guideline on long-term management of children with bronchopulmonary dysplasia. Eur Respir J 2020; 55(1).
- Doyle LW: Evaluation of neonatal intensive care for extremely-low-birth-weight infants. Semin Fetal Neonatal Med 2006; 11(2): 139-145.
- Gortner L, Misselwitz B, Milligan D, members of the MRG, et al: Rates of bronchopulmonary dysplasia in very preterm neonates in Europe: results from the MOSAIC cohort. Neonatology 2011; 99(2): 112-117.
- Stoll BJ, Hansen NI, Bell EF, Eunice Kennedy Shriver National Institute of Child H, Human Development Neonatal Research N, et al: Neonatal outcomes of extremely preterm infants from the NICHD Neonatal Research Network. Pediatrics 2010; 126(3): 443-456.
- Vachon E, Bourbonnais Y, Bingle CD, et al: Anti-inflammatory effect of pre-elafin in lipopolysaccharide-induced acute lung inflammation. Biol Chem 2002; 383(7-8): 1249-1256.
- Van Marter LJ, Pagano M, Allred EN, et al: Rate of bronchopulmonary dysplasia as a function of neonatal intensive care practices. J Pediatr 1992; 120(6): 938-946.
- Ehrenkranz RA, Walsh MC, Vohr BR, National Institutes of Child H, Human Development Neonatal Research N, et al: Validation of the National Institutes of Health consensus definition of bronchopulmonary dysplasia. Pediatrics 2005; 116(6): 1353-1360.
- Johnson AH, Peacock JL, Greenough A, United Kingdom Oscillation Study G, et al: High-frequency oscillatory ventilation for the prevention of chronic lung disease of prematurity. N Engl J Med 2002; 347(9): 633-642.
- Chawanpaiboon S, Vogel JP, Moller AB, et al: Global, regional, and national estimates of levels of preterm birth in 2014; a systematic review and modelling analysis. Lancet Glob Health 2019; 7(1): e37-e46.
- Clyman R, Cassady G, Kirklin JK, et al: The role of patent ductus arteriosus ligation in bronchopulmonary dysplasia: reexamining a randomized controlled trial. J Pediatr 2009; 154(6): 873-876.
- Korhonen P, Tammela O, Koivisto AM, et al: Frequency and risk factors in bronchopulmonary dysplasia in a cohort of very low birth weight infants. Early Hum Dev 1999; 54(3): 245-258.
- Kramer BW: Antenatal inflammation and lung injury: prenatal origin of neonatal disease. J Perinatol 2008; 28 Suppl 1: S21-27.
- Mittendorf R, Covert R, Montag AG, et al: Special relationships between fetal inflammatory response syndrome and bronchopulmonary dysplasia in neonates. J Perinat Med 2005; 33(5): 428-434.
- Oh W, Poindexter BB, Perritt R, Neonatal Research N, et al: Association between fluid intake and weight loss during the first ten days of life and risk of bronchopulmonary dysplasia in extremely low birth weight infants. J Pediatr 2005; 147(6): 786-790.
- Stevens TP, Harrington EW, Blennow M, Soll RF: Early surfactant administration with brief ventilation vs. selective surfactant and continued mechanical ventilation for preterm infants with or at risk for respiratory distress syndrome. Cochrane Database Syst Rev 2007(4); CD003063.
- Biniwale MA, Ehrenkranz RA: The role of nutrition in the prevention and management of bronchopulmonary dysplasia. Semin Perinatol 2006; 30(4): 200-208.
- Shenai JP, Chytil F, Stahlman MT: Vitamin A status of neonates with bronchopulmonary dysplasia. Pediatr Res 1985; 19(2): 185-188.
- Watterberg KL, Scott SM: Evidence of early adrenal insufficiency in babies who develop bronchopulmonary dysplasia. Pediatrics 1995; 95(1): 120-125.
- Bose C, Van Marter LJ, Laughon M, Extremely Low Gestational Age Newborn Study I, et al: Fetal growth restriction and chronic lung disease among infants born before the 28th week of gestation. Pediatrics 2009; 124(3): e450-458.
- Regev RH, Lusky A, Dolfin T, Israel Neonatal N, et al: Excess mortality and morbidity among small-for-gestational-age premature infants: a population-based study. J Pediatr 2003; 143(2): 186-191.
- Reiss I, Landmann E, Heckmann M, et al: Increased risk of bronchopulmonary dysplasia and increased mortality in very preterm infants being small for gestational age. Arch Gynecol Obstet 2003; 269(1): 40-44.
- Rieger-Fackeldey E, Schulze A, Pohlandt F, et al: Short-term outcome in infants with a birthweight less than 501 grams. Acta Paediatr 2005; 94(2): 211-216.
- Walsh MC, Yao Q, Horbar JD, et al: Changes in the use of postnatal steroids for bronchopulmonary dysplasia in 3 large neonatal networks. Pediatrics 2006; 118(5): e1328-1335.
- Rozance PJ, Seedorf GJ, Brown A, et al: Intrauterine growth restriction decreases pulmonary alveolar and vessel growth and causes pulmonary artery endothelial cell dysfunction in vitro in fetal sheep. Am J Physiol Lung Cell Mol Physiol 2011; 301(6): L860-871.
- Gilliland FD, Berhane K, McConnell R, et al: Maternal smoking during pregnancy, environmental tobacco smoke exposure and childhood lung function. Thorax 2000; 55(4): 271-276.
- Vogt Isaksen C: Maternal smoking, intrauterine growth restriction, and placental apoptosis. Pediatr Dev Pathol 2004; 7(5): 433-442.
- Bhandari V, Bizzarro MJ, Shetty A, Neonatal Genetics Study G, et al: Familial and genetic susceptibility to major neonatal morbidities in preterm twins. Pediatrics 2006; 117(6): 1901-1906.
- Hallman M, Haataja R: Genetic influences and neonatal lung disease. Semin Neonatol 2003; 8(1): 19-27.
- Hilgendorff A, Heidinger K, Pfeiffer A, et al: Association of polymorphisms in the mannose-binding lectin gene and pulmonary morbidity in preterm infants. Genes Immun 2007; 8(8): 671-677.
- Poggi C, Giusti B, Vestri A, et al: Genetic polymorphisms of antioxidant enzymes in preterm infants. J Matern Fetal Neonatal Med 2012; 25 Suppl 4: 131-134.
- Binet ME, Bujold E, Lefebvre F, Canadian Neonatal N, et al: Role of gender in morbidity and mortality of extremely premature neonates. Am J Perinatol 2012; 29(3): 159-166.
- Trotter A, Maier L, Kron M, Pohlandt F: Effect of oestradiol and progesterone replacement on bronchopulmonary dysplasia in extremely preterm infants. Arch Dis Child Fetal Neonatal Ed 2007; 92(2): F94-98.
- Vrijlandt EJ, Gerritsen J, Boezen HM, Duiverman EJ, Dutch P-CSG: Gender differences in respiratory symptoms in 19-year-old adults born preterm. Respir Res 2005; 6: 117.
- Wadon M, Modi N, Wong HS, et al: Recent advances in the genetics of preterm birth. Ann Hum Genet 2020; 84(3): 205-213.
- Fraser J, Walls M, McGuire W: Respiratory complications of preterm birth. BMJ 2004; 329(7472): 962-965.
- Wang H, St Julien KR, Stevenson DK, et al: A genome-wide association study (GWAS) for bronchopulmonary dysplasia. Pediatrics 2013; 132(2): 290-297.
- Lommatzsch ST, Aris R: Genetics of cystic fibrosis. Semin Respir Crit Care Med 2009; 30(5): 531-538.
- Sztrymf B, Coulet F, Girerd B, et al: Clinical outcomes of pulmonary arterial hypertension in carriers of BMPR2 mutation. Am J Respir Crit Care Med 2008; 177(12): 1377-1383.
- Austin ED, Loyd JE: The genetics of pulmonary arterial hypertension. Circ Res 2014; 115(1): 189-202.
- Husain AN, Siddiqui NH, Stocker JT: Pathology of arrested acinar development in postsurfactant bronchopulmonary dysplasia. Hum Pathol 1998; 29(7): 710-717.
- Lopez E, Mathlouthi J, Lescure S, et al: Capnography in spontaneously breathing preterm infants with bronchopulmonary dysplasia. Pediatr Pulmonol 2011; 46(9): 896-902.
- Stoll BJ, Gordon T, Korones SB, et al: Early-onset sepsis in very low birth weight neonates: a report from the National Institute of Child Health and Human Development Neonatal Research Network. J Pediatr 1996; 129(1): 72-80.
- Watterberg KL, Demers LM, Scott SM, Murphy S: Chorioamnionitis and early lung inflammation in infants in whom bronchopulmonary dysplasia develops. Pediatrics 1996; 97(2): 210-215.
- Yoon BH, Romero R, Jun JK, et al: Amniotic fluid cytokines (interleukin-6, tumor necrosis factor-alpha, interleukin-1 beta, and interleukin-8) and the risk for the development of bronchopulmonary dysplasia. Am J Obstet Gynecol 1997; 177(4): 825-830.
- Speer CP: Inflammation and bronchopulmonary dysplasia: a continuing story. Semin Fetal Neonatal Med 2006; 11(5): 354-362.
- Todd DA, Earl M, Lloyd J, Greenberg M, John E: Cytological changes in endotracheal aspirates associated with chronic lung disease. Early Hum Dev 1998; 51(1): 13-22.
- Ibrahim J, Garantziotis S, Savani R: Updates on Neonatal Chronic Lung Disease 2020, Chapter 9 – The Inflammation Superhighway: Tolls, Signals, and Pathways to Bronchopulmonary Dysplasia. 131-150.
- Atochina-Vasserman EN, Bates SR, Zhang P, et al: Early alveolar epithelial dysfunction promotes lung inflammation in a mouse model of Hermansky-Pudlak syndrome. Am J Respir Crit Care Med 2011; 184(4): 449-458.
- Hilgendorff A, Parai K, Ertsey R, et al: Inhibiting lung elastase activity enables lung growth in mechanically ventilated newborn mice. Am J Respir Crit Care Med 2011; 184(5): 537-546.
- Stoll BJ, Hansen N, Fanaroff AA, et al: Changes in pathogens causing early-onset sepsis in very-low-birth-weight infants. N Engl J Med 2002; 347(4): 240-247.
- Olszak T, An D, Zeissig S, et al: Microbial exposure during early life has persistent effects on natural killer T cell function. Science 2012; 336(6080): 489-493.
- Bose CL, Laughon MM, Allred EN, Investigators ES, et al: Systemic inflammation associated with mechanical ventilation among extremely preterm infants. Cytokine 2013; 61(1): 315-322.
- Jobe AH, Kallapur SG: Long term consequences of oxygen therapy in the neonatal period. Semin Fetal Neonatal Med 2010; 15(4): 230-235.
- Kroon AA, Wang J, Huang Z, et al: Inflammatory response to oxygen and endotoxin in newborn rat lungs ventilated with low tidal volume. Pediatr Res 2010; 68(1): 63-69.
- Polglase GR, Hillman NH, Ball MK, et al: Lung and systemic inflammation in preterm lambs on continuous positive airway pressure or conventional ventilation. Pediatr Res 2009; 65(1): 67-71.
- Kunzmann S, Speer CP, Jobe AH, Kramer BW: Antenatal inflammation induced TGF-beta1 but suppressed CTGF in preterm lungs. Am J Physiol Lung Cell Mol Physiol 2007; 292(1): L223-231.
- Kazzi SN, Kim UO, Quasney MW, Buhimschi I: Polymorphism of tumor necrosis factor-alpha and risk and severity of bronchopulmonary dysplasia among very low birth weight infants. Pediatrics 2004; 114(2): e243-248.
- Nold MF, Mangan NE, Rudloff I, et al: Interleukin-1 receptor antagonist prevents murine bronchopulmonary dysplasia induced by perinatal inflammation and hyperoxia. Proc Natl Acad Sci U S A 2013; 110(35): 14384-14389.
- Ibrahim JG, Stavros; Savani, Rashmin: The Inflammation Superhighway: Tolls, Signals, and Pathways to Bronchopulmonary Dysplasia. In: Kallapur SP, Gloria, ed. Updates on Neonatal Chronic Lung Disease. Elsevier 2020; 131-150.
- Lechner AJ, Driver IH, Lee J, et al: Recruited monocytes and type 2 immunity promote lung regeneration following pneumonectomy. Cell Stem Cell 2017; 21(1): 120-134 e127.
- McQuattie-Pimentel AC, Budinger GRS, Ballinger MN: Monocyte-derived alveolar macrophages: the dark side of lung repair? Am J Respir Cell Mol Biol 2018; 58(1): 5-6.
- Olin A, Henckel E, Chen Y, et al: Stereotypic Immune System Development in Newborn Children. Cell 2018; 174(5): 1277-1292 e1214.
- Zaslona Z, Przybranowski S, Wilke C, et al: Resident alveolar macrophages suppress, whereas recruited monocytes promote, allergic lung inflammation in murine models of asthma. J Immunol 2014; 193(8): 4245-4253.
- Yamada M, Fujino N, Ichinose M: Inflammatory responses in the initiation of lung repair and regeneration: their role in stimulating lung resident stem cells. Inflamm Regen 2016; 36: 15.
- Bose CL, Dammann CE, Laughon MM: Bronchopulmonary dysplasia and inflammatory biomarkers in the premature neonate. Arch Dis Child Fetal Neonatal Ed 2008; 93(6): F455-461.
- Rose MJ, Stenger MR, Joshi MS, et al: Inhaled nitric oxide decreases leukocyte trafficking in the neonatal mouse lung during exposure to >95% oxygen. Pediatr Res 2010; 67(3): 244-249.
- Vento G, Tirone C, Lulli P, et al: Bronchoalveolar lavage fluid peptidomics suggests a possible matrix metalloproteinase-3 role in bronchopulmonary dysplasia. Intensive Care Med 2009; 35(12): 2115-2124.
- Watterberg KL, Carmichael DF, Gerdes JS, et al: Secretory leukocyte protease inhibitor and lung inflammation in developing bronchopulmonary dysplasia. J Pediatr 1994; 125(2): 264-269.
- Weinberger B, Anwar M, Henien S, et al: Association of lipid peroxidation with antenatal betamethasone and oxygen radial disorders in preterm infants. Biol Neonate 2004; 85(2): 121-127.
- Collard KJ, Godeck S, Holley JE, Quinn MW: Pulmonary antioxidant concentrations and oxidative damage in ventilated premature babies. Arch Dis Child Fetal Neonatal Ed 2004; 89(5): F412-416.
- Filippone M, Bonetto G, Corradi M, et al: Evidence of unexpected oxidative stress in airways of adolescents born very pre-term. Eur Respir J 2012; 40(5): 1253-1259.
- Hennessy EM, Bracewell MA, Wood N, Group EPS, et al: Respiratory health in pre-school and school age children following extremely preterm birth. Arch Dis Child 2008; 93(12): 1037-1043.
- Merritt TA, Deming DD, Boynton BR: The ‘new’ bronchopulmonary dysplasia: challenges and commentary. Semin Fetal Neonatal Med 2009; 14(6): 345-357.
- Buczynski BW, Yee M, Paige Lawrence B, O’Reilly MA: Lung development and the host response to influenza A virus are altered by different doses of neonatal oxygen in mice. Am J Physiol Lung Cell Mol Physiol 2012; 302(10): L1078-1087.
- Belik J, Jankov RP, Pan J, Tanswell AK: Chronic O2 exposure enhances vascular and airway smooth muscle contraction in the newborn but not adult rat. J Appl Physiol (1985) 2003; 94(6): 2303-2312.
- Yee M, White RJ, Awad HA, Bates WA, et al: Neonatal hyperoxia causes pulmonary vascular disease and shortens life span in aging mice. Am J Pathol 2011; 178(6): 2601-2610.
- Balasubramaniam V, Mervis CF, Maxey AM, et al: Hyperoxia reduces bone marrow, circulating, and lung endothelial progenitor cells in the developing lung: implications for the pathogenesis of bronchopulmonary dysplasia. Am J Physiol Lung Cell Mol Physiol 2007; 292(5): L1073-1084.
- Londhe VA, Sundar IK, Lopez B, et al: Hyperoxia impairs alveolar formation and induces senescence through decreased histone deacetylase activity and up-regulation of p21 in neonatal mouse lung. Pediatr Res 2011; 69(5 Pt 1): 371-377.
- Nanduri J, Makarenko V, Reddy VD, et al: Epigenetic regulation of hypoxic sensing disrupts cardiorespiratory homeostasis. Proc Natl Acad Sci U S A 2012; 109(7): 2515-2520.
- Jankov RP, Keith Tanswell A: Growth factors, postnatal lung growth and bronchopulmonary dysplasia. Paediatr Respir Rev 2004; 5 Suppl A: S265-275.
- Alejandre-Alcazar MA, Kwapiszewska G, Reiss I, et al: Hyperoxia modulates TGF-beta/BMP signaling in a mouse model of bronchopulmonary dysplasia. Am J Physiol Lung Cell Mol Physiol 2007; 292(2): L537-549.
- Chao CM, Moiseenko A, Kosanovic D, et al: Impact of Fgf10 deficiency on pulmonary vasculature formation in a mouse model of bronchopulmonary dysplasia. Hum Mol Genet 2019; 28(9): 1429-1444.
- Li C, Smith SM, Peinado N, et al: WNT5a-ROR Signaling Is Essential for Alveologenesis. Cells 2020; 9(2).
- Oak P, Hilgendorff A: The BPD trio? Interaction of dysregulated PDGF, VEGF, and TGF signaling in neonatal chronic lung disease. Mol Cell Pediatr 2017; 4(1): 11.
- Sucre JM, Wilkinson D, Vijayaraj P, et al: A three-dimensional human model of the fibroblast activation that accompanies bronchopulmonary dysplasia identifies Notch-mediated pathophysiology. Am J Physiol Lung Cell Mol Physiol 2016; 310(10): L889-898.
- Sucre JMS, Deutsch GH, Jetter CS, et al: A Shared Pattern of beta-Catenin Activation in Bronchopulmonary Dysplasia and Idiopathic Pulmonary Fibrosis. Am J Pathol 2018; 188(4): 853-862.
- Iosef C, Alastalo TP, Hou Y, et al: Inhibiting NF-kappaB in the developing lung disrupts angiogenesis and alveolarization. Am J Physiol Lung Cell Mol Physiol 2012; 302(10): L1023-1036.
- Didon L, Roos AB, Elmberger GP, et al: Lung-specific inactivation of CCAAT/enhancer binding protein alpha causes a pathological pattern characteristic of COPD. Eur Respir J 2010; 35(1): 186-197.
- Alvira CM, Abate A, Yang G, et al: Nuclear factor-kappaB activation in neonatal mouse lung protects against lipopolysaccharide-induced inflammation. Am J Respir Crit Care Med 2007; 175(8): 805-815.
- Yang G, Abate A, George AG, et al: Maturational differences in lung NF-kappaB activation and their role in tolerance to hyperoxia. J Clin Invest 2004; 114(5): 669-678.
- De Paepe ME, Greco D, Mao Q: Angiogenesis-related gene expression profiling in ventilated preterm human lungs. Exp Lung Res 2010; 36(7): 399-410.
- De Paepe ME, Mao Q, Powell J, et al: Growth of pulmonary microvasculature in ventilated preterm infants. Am J Respir Crit Care Med 2006; 173(2): 204-211.
- Thebaud B: Angiogenesis in lung development, injury and repair: implications for chronic lung disease of prematurity. Neonatology 2007; 91(4): 291-297.
- Ito Y, Betsuyaku T, Nagai K, et al: Expression of pulmonary VEGF family declines with age and is further down-regulated in lipopolysaccharide (LPS)-induced lung injury. Exp Gerontol 2005; 40(4): 315-323.
- Vyas-Read S, Shaul PW, Yuhanna IS, Willis BC: Nitric oxide attenuates epithelial-mesenchymal transition in alveolar epithelial cells. Am J Physiol Lung Cell Mol Physiol 2007; 293(1): L212-221.
- Bland RD, Ling CY, Albertine KH, et al: Pulmonary vascular dysfunction in preterm lambs with chronic lung disease. Am J Physiol Lung Cell Mol Physiol 2003; 285(1): L76-85.
- Kamba T, Tam BY, Hashizume H, Haskell A, et al: VEGF-dependent plasticity of fenestrated capillaries in the normal adult microvasculature. Am J Physiol Heart Circ Physiol 2006; 290(2): H560-576.
- Kinsella JP, Greenough A, Abman SH: Bronchopulmonary dysplasia. Lancet 2006; 367(9520): 1421-1431.
- Steinhorn RH: Neonatal pulmonary hypertension. Pediatr Crit Care Med 2010; 11(2 Suppl): S79-84.
- Torday JS, Rehan VK: Mechanotransduction determines the structure and function of lung and bone: a theoretical model for the pathophysiology of chronic disease. Cell Biochem Biophys 2003; 37(3): 235-246.
- Mizikova I, Morty RE: The Extracellular Matrix in Bronchopulmonary Dysplasia: Target and Source. Front Med (Lausanne) 2015; 2: 91.
- Bruce MC, Schuyler M, Martin RJ, et al: Risk factors for the degradation of lung elastic fibers in the ventilated neonate. Implications for impaired lung development in bronchopulmonary dysplasia. Am Rev Respir Dis 1992; 146(1): 204-212.
- Bruce MC, Wedig KE, Jentoft N, et al: Altered urinary excretion of elastin cross-links in premature infants who develop bronchopulmonary dysplasia. Am Rev Respir Dis 1985; 131(4): 568-572.
- Merritt TA, Cochrane CG, Holcomb K, et al: Elastase and alpha 1-proteinase inhibitor activity in tracheal aspirates during respiratory distress syndrome. Role of inflammation in the pathogenesis of bronchopulmonary dysplasia. J Clin Invest 1983; 72(2): 656-666.
- Tenholder MF, Rajagopal KR, Phillips YY, et al: Urinary desmosine excretion as a marker of lung injury in the adult respiratory distress syndrome. Chest 1991; 100(5): 1385-1390.
- Lukkarinen H, Hogmalm A, Lappalainen U, Bry K: Matrix metalloproteinase-9 deficiency worsens lung injury in a model of bronchopulmonary dysplasia. Am J Respir Cell Mol Biol 2009; 41(1): 59-68.
- Pierce RA, Albertine KH, Starcher BC, et al: Chronic lung injury in preterm lambs: disordered pulmonary elastin deposition. Am J Physiol 1997; 272(3 Pt 1): L452-460.
- Thibeault DW, Mabry SM, Ekekezie, II, Truog WE: Lung elastic tissue maturation and perturbations during the evolution of chronic lung disease. Pediatrics 2000; 106(6): 1452-1459.
- Thibeault DW, Mabry SM, et al: Collagen scaffolding during development and its deformation with chronic lung disease. Pediatrics 2003; 111(4 Pt 1): 766-776.
- Bonvillain RW, Danchuk S, Sullivan DE, et al: A nonhuman primate model of lung regeneration: detergent-mediated decellularization and initial in vitro recellularization with mesenchymal stem cells. Tissue Eng Part A 2012; 18(23-24): 2437-2452.
- Jensen T, Roszell B, Zang F, et al: A rapid lung de-cellularization protocol supports embryonic stem cell differentiation in vitro and following implantation. Tissue Eng Part C Methods 2012; 18(8): 632-646.
- Förster K, Ertl-Wagner B, Ehrhardt H, et al: Altered relaxation times in MRI indicate bronchopulmonary dysplasia. Thorax 2019; thoraxjnl-2018-212384.
- Oda K, Ishimoto H, Yatera K, et al: High-resolution CT scoring system-based grading scale predicts the clinical outcomes in patients with idiopathic pulmonary fibrosis. Respir Res 2014; 15: 10.
- Walkup LL, Tkach JA, Higano NS, et al: Quantitative magnetic resonance imaging of bronchopulmonary dysplasia in the neonatal intensive care unit environment. Am J Respir Crit Care Med 2015; 192(10): 1215-1222.
- Washko GR: Diagnostic imaging in COPD. Semin Respir Crit Care Med 2010; 31(3): 276-285.
- Weatherley ND, Eaden JA, Stewart NJ, et al: Experimental and quantitative imaging techniques in interstitial lung disease. Thorax 2019; 74(6): 611-619.
- Capaldi DP, Zha N, Guo F, et al: Pulmonary imaging biomarkers of gas trapping and emphysema in COPD: (3)He MR imaging and CT parametric response maps. Radiology 2016; 279(2): 597-608.
- De Boeck K, Derichs N, Fajac I, Group EDNW, EuroCare CFWPGoCFd, et al: New clinical diagnostic procedures for cystic fibrosis in Europe. J Cyst Fibros 2011; 10 Suppl 2: S53-66.
- Sileo C, Corvol H, Boelle PY, et al: HRCT and MRI of the lung in children with cystic fibrosis: comparison of different scoring systems. J Cyst Fibros 2014; 13(2): 198-204.
- Tulek B, Kivrak AS, Ozbek S, et al: Phenotyping of chronic obstructive pulmonary disease using the modified Bhalla scoring system for high-resolution computed tomography. Can Respir J 2013; 20(2): 91-96.
- Jobe AH, Ikegami M: Prevention of bronchopulmonary dysplasia. Curr Opin Pediatr 2001; 13(2): 124-129.
- Newman JB, DeBastos AG, Batton D, Raz S: Neonatal respiratory dysfunction and neuropsychological performance at the preschool age: a study of very preterm infants with bronchopulmonary dysplasia. Neuropsychology 2011; 25(5): 666-678.
- Bolton CE, Bush A: Coming now to a chest clinic near you. Thorax 2013; 68(8): 707-708.
- Bolton CE, Bush A, Hurst JR, et al: Lung consequences in adults born prematurely. Thorax 2015; 70(6): 574-580.
- May C, Kennedy C, Milner AD, et al: Lung function abnormalities in infants developing bronchopulmonary dysplasia. Arch Dis Child 2011; 96(11): 1014-1019.
- Miller MR, Pedersen OF, Lange P, Vestbo J: Improved survival prediction from lung function data in a large population sample. Respir Med 2009; 103(3): 442-448.
- Hilgendorff A, Reiss I, Gortner L, et al: Impact of airway obstruction on lung function in very preterm infants at term. Pediatr Crit Care Med 2008; 9(6): 629-635.
- Greenough A, Alexander J, Burgess S, et al: Preschool healthcare utilization related to home oxygen status. Arch Dis Child Fetal Neonatal Ed 2006; 91(5): F337-341.
- Greenough A, Alexander J, Burgess S, et al: Home oxygen status and rehospitalization and primary care requirements of infants with chronic lung disease. Arch Dis Child 2002; 86(1): 40-43.
- Doyle LW, Cheung MM, Ford GW, et al: Birth weight <1501 g and respiratory health at age 14. Arch Dis Child 2001; 84(1): 40-44.
- Greenough A, Cox S, Alexander J, et al: Health care utilization of infants with chronic lung disease, related to hospitalization for RSV infection. Arch Dis Child 2001; 85(6): 463-468.
- Broughton S, Roberts A, Fox G, et al: Prospective study of healthcare utilization and respiratory morbidity due to RSV infection in prematurely born infants. Thorax 2005; 60(12): 1039-1044.
- Baraldi E, Carraro S, Filippone M: Bronchopulmonary dysplasia: definitions and long-term respiratory outcome. Early Hum Dev 2009; 85(10 Suppl): 1-3.
- Greenough A, Limb E, Marston L, et al: Risk factors for respiratory morbidity in infancy after very premature birth. Arch Dis Child Fetal Neonatal Ed 2005; 90(4): F320-323.
- Gross SJ, Iannuzzi DM, Kveselis DA, Anbar RD: Effect of preterm birth on pulmonary function at school age: a prospective controlled study. J Pediatr 1998; 133(2): 188-192.
- Broughton S, Thomas MR, Marston L, et al: Very prematurely born infants wheezing at follow-up: lung function and risk factors. Arch Dis Child 2007; 92(9): 776-780.
- Pelkonen AS, Hakulinen AL, Turpeinen M: Bronchial lability and responsiveness in school children born very preterm. Am J Respir Crit Care Med 1997; 156(4 Pt 1): 1178-1184.
- Yuksel B, Greenough A: Relationship of symptoms to lung function abnormalities in preterm infants at follow-up. Pediatr Pulmonol 1991; 11(3): 202-206.
- Welsh L, Kirkby J, Lum S, Odendaal D, Group EPS, et al: The EPICure study: maximal exercise and physical activity in school children born extremely preterm. Thorax 2010; 65(2): 165-172.
- Doyle LW, Faber B, Callanan C, et al: Bronchopulmonary dysplasia in very low birth weight subjects and lung function in late adolescence. Pediatrics 2006; 118(1): 108-113.
- Greenough A, Dimitriou G, Bhat RY, et al: Lung volumes in infants who had mild to moderate bronchopulmonary dysplasia. Eur J Pediatr 2005; 164(9): 583-586.
- Hjalmarson O, Sandberg KL: Lung function at term reflects severity of bronchopulmonary dysplasia. J Pediatr 2005; 146(1): 86-90.
- Hurst JR, Beckmann J, Ni Y, et al: Respiratory and Cardiovascular Outcomes in Survivors of Extremely Preterm Birth at 19 Years. Am J Respir Crit Care Med 2020; 202(3): 422-432.
- Northway WH, Jr, Moss RB, Carlisle KB, et al: Late pulmonary sequelae of bronchopulmonary dysplasia. N Engl J Med 1990; 323(26): 1793-1799.
- Vollsaeter M, Roksund OD, Eide GE, et al: Lung function after preterm birth: development from mid-childhood to adulthood. Thorax 2013; 68(8): 767-776.
- Bolton CE, Bush A, Hurst JR, et al: Are early life factors considered when managing respiratory disease? A British Thoracic Society survey of current practice. Thorax 2012; 67(12): 1110.
- Crump C: Medical history taking in adults should include questions about preterm birth. BMJ 2014; 349: g4860.
- Baraldi E, Filippone M: Chronic lung disease after premature birth. N Engl J Med 2007; 357(19): 1946-1955.
- Filippone M, Carraro S, Baraldi E: From BPD to COPD? The hypothesis is intriguing but we lack lung pathology data in humans. Eur Respir J 2010; 35(6): 1419-1420; author reply 1420.
- Anderson PJ, Doyle LW: Neurodevelopmental outcome of bronchopulmonary dysplasia. Semin Perinatol 2006; 30(4): 227-232.
- Bal MP, de Vries WB, van Oosterhout MF, et al: Long-term cardiovascular effects of neonatal dexamethasone treatment: hemodynamic follow-up by left ventricular pressure-volume loops in rats. J Appl Physiol (1985) 2008; 104(2): 446-450.
- Kamphuis PJ, de Vries WB, Bakker JM, et al: Reduced life expectancy in rats after neonatal dexamethasone treatment. Pediatr Res 2007; 61(1): 72-76.
InFo PNEUMOLOGY & ALLERGOLOGY 2021; 3(2): 10-15.